Strongly nonlinear internal solitary waves are a ubiquitous feature of the coastal ocean. They have been observed in moored records, from shipboard measurements and remotely by radar and aerial photography. Strong shear generated by these waves creates conditions favorable for instabilities, producing elevated turbulent dissipation and mixing. Since this mixing is often concentrated at the pycnocline, waves may be a fundamental contributor to vertical nutrient and heat flux over the shelf.
In the header image, acoustical backscatter in a propagating internal wave highlights a sequence of rollups identical in nature to Kelvin–Helmholtz instabilities observed in the laboratory and in small-scale simulations. The vertical scale of the largest is more than 10 m, and the horizontal scale (in the direction of wave propagation) is about 50 m. Toward the trailing edge of the wave, the rollups become less coherent but contribute a greater backscatter signal, suggesting breakdown to turbulence. At greater depth, denoted by arrows, are two more layers of bright backscatter. These are presumably the same phenomenon, but smaller scale. Hence, even the fine echosounder resolution does not permit a clear depiction of these deeper rollups. (from Moum et.al., 2003).
Below is an example of three waves observed using shipboard acoustics. (a) acoustic backscatter, (b) horizontal velocity, u, and (c) vertical velocity, w. The waves are propagating from right to left. We recognize these as waves that depress the scattering layers down as w is first negative on the leading edge of the waves and then positive after the surface pulse passes. The excitation of the return bottom pulse is required by conservation of fluid volume (mass). (from Shroyer et.al., 2011)
These waves are frequently observed visually at the sea surface where the alternating converging and diverging surfaces at leading and trailing edges of the waves act to enhance and reduce small scale surface waves as seen in these lines of alternating bands of rough/smooth sea surface over the NJ continental shelf (photo below). This also leads to a radar signature (usually X-band / 3 cm) that can be sampled and used as a wave tracking measurement from ship or satellite.
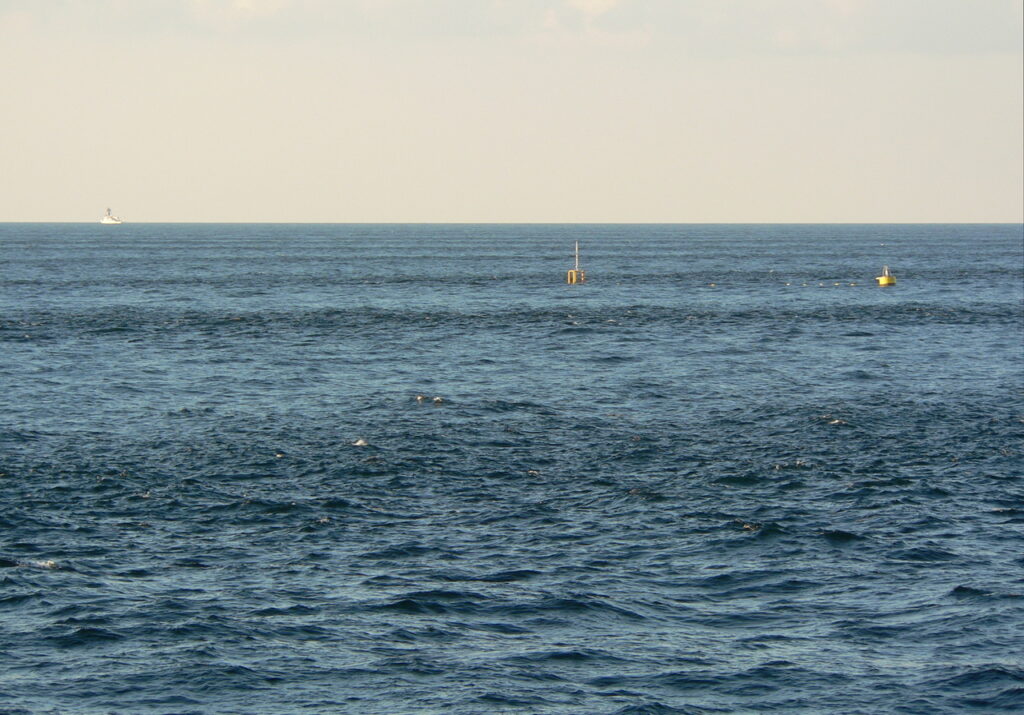
_________________________________________________________________
Structure and generation of turbulence at interfaces strained by internal solitary waves propagating shoreward over the continental shelf, J. Phys. Oceanogr ., 33, 2093-2112, doi: https://doi.org/10.1175/1520-0485(2003)033%3C2093:SAGOTA%3E2.0.CO;2, 2003 (J.N. Moum, D.M. Farmer, W.D. Smyth, L. Armi and S. Vagle).
Internal solitary waves of elevation advancing on a sloping shelf, Geophys. Res. Lett . 30, NO. 20, 2045, doi: https://doi.org/10.1029/2003GL017706, 2003 (J. M. Klymak and J.N. Moum).
River plumes as a source of large-amplitude internal waves in the coastal ocean, Nature, 437, 400-403, doi: https://doi.org/10.1038/nature03936, 2005 (J.D. Nash and J.N. Moum).
The pressure disturbance of a nonlinear internal wave train, J. Fluid Mech, 558, 153-177, doi: https://doi.org/10.1017/S0022112006000036, 2006. (J.N. Moum and W.D. Smyth)
Dissipative losses in nonlinear internal waves propagating across the continental shelf, J. Phys. Oceanogr., 37(7), 1989-1995, doi: https://doi.org/10.1175/JPO3091.1, 2007 (J.N. Moum, D.M. Farmer, E.L. Shroyer, W.D. Smyth and L. Armi).
Energy transport by nonlinear internal waves, J. Phys. Oceanogr., 37(7), 1968-1988, doi: https://doi.org/10.1175/JPO3094.1, 2007 (J.N. Moum, J.M. Klymak, J.D. Nash, A. Perlin and W.D. Smyth).
Seafloor pressure measurements of nonlinear internal waves, J. Phys. Oceanogr., 38(2), 481-491, doi: https://doi.org/10.1175/2007JPO3736.1, 2008 (J.N. Moum and J.D. Nash).
Observations of polarity reversal in shoaling nonlinear internal waves, J. Phys. Oceanogr., 39, 691-701, doi: https://doi.org/10.1175/2008JPO3953.1, 2009 (E.L. Shroyer, J.N. Moum, and J.D. Nash).
Mode 2 waves on the continental shelf: Ephemeral components of the nonlinear internal wavefield. J. Geophys. Res., 115, C07001, doi: https://doi.org/10.1029/2009JC005605, 2010 (E.L. Shroyer, J.N. Moum, and J.D. Nash).
Vertical heat flux and lateral mass transport in nonlinear internal waves, Geophys. Res. Lett., 37, L08601, doi: https://doi.org/10.1029/2010GL042715, 2010 (E.L. Shroyer, J.N. Moum, and J.D. Nash).
Energy transformations and dissipation of nonlinear internal waves over New Jersey’s continental shelf. Nonlinear Proc. Geophys., 17, 345-360, doi: https://doi.org/10.5194/npg-17-345-2010, 2010 (E.L. Shroyer, J.N. Moum, and J.D. Nash).
Nonlinear internal waves over New Jersey’s continental shelf. J. Geophys. Res., 116, C03022, doi: https://doi.org/10.1029/2010JC006332, 2011 (E.L. Shroyer, J.N. Moum and J.D. Nash).
On the potential for automated realtime detection of nonlinear internal waves from seafloor pressure measurements, Appl. Ocean Res., 33, 275-285, doi: https://doi.org/10.1016/j.apor.2011.07.007, 2011 (U. Stoeber and J.N. Moum)
Horizontal variability of high-frequency nonlinear internal waves in Massachusetts Bay detected by an array of seafloor pressure sensors, J. Geophys. Res., 121, 5587-5607, https://doi.org/10.1002/2016JC011866, 2016 (J.A. Thomas, JA. Lerczak and J.N. Moum).